How To Advance Continuous Bioprocessing Using Continuous Countercurrent Tangential Chromatography
By Andrew Zydney, Ph.D., Department of Chemical Engineering, The Pennsylvania State University
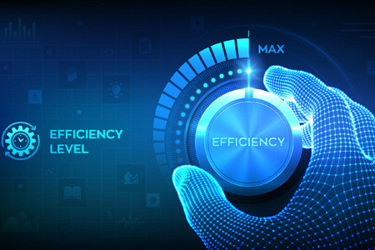
Several recent publications have examined the potential benefits of continuous biomanufacturing, including reductions in cost of goods, greater flexibility in developing multiproduct facilities, and improved product quality due to the shorter and more uniform residence times.1,2 For example, Walther et al.3 concluded that an integrated continuous biomanufacturing platform could reduce processing costs (net present value) by 55% relative to conventional batch operations for production of monoclonal antibodies (mAbs) with even greater cost reductions estimated for other recombinant protein products. Continuous bioreactors have been shown to provide much higher productivity than corresponding fed-batch systems with better product quality and reduced intra-lot heterogeneity.4
Although continuous perfusion bioreactors have been used in commercial biomanufacturing for more than 25 years, the implementation of continuous downstream processes is much more limited.5 One of the key challenges is in the initial capture step employing bind-and-elute chromatography, e.g., Protein A affinity chromatography for capture of mAbs. Bind-and-elute chromatography is inherently a batch process: Column washing cannot start until the resin is fully loaded with bound product, elution cannot start until washing is complete, and stripping and then regeneration cannot begin until product recovery is finished. A number of multi-column chromatography processes have been developed that provide continuous product loading, but these systems operate cyclically with the concentration and pH/conductivity of the eluted product stream varying in a periodic fashion. This not only complicates the integration of these systems with subsequent downstream unit operations, but it also creates challenges for the development of appropriate process control strategies that would be required for long-term operation of a fully integrated continuous manufacturing process.
Figure 1: Schematic of continuous countercurrent tangential chromatography (CCTC) for impurity removal (filled ovals) from a bound monoclonal antibody (Y-shaped). From Zydney (2021).7
Continuous Countercurrent Tangential Chromatography
Continuous countercurrent tangential chromatography (CCTC) addresses the inherent limitations of column-based systems by conducting the chromatographic operations (binding, washing, elution, stripping, and regeneration) on a flowing slurry, with inline static mixers used to ensure good contacting between the resin slurry and the clarified cell culture fluid/required buffers.6 Hollow fiber membrane modules are then used for the fluid-resin separation, e.g., to wash impurities like host cell proteins away from the resin-bound product that is fully retained by the membrane (Figure 1). One of the unique characteristics of CCTC is that the static mixer-membrane combinations are arranged in a countercurrent configuration to maximize the degree of product purification and yield while minimizing the amount of buffer needed for the process.6 This type of countercurrent staging is widely used in chemical manufacturing, e.g., in distillation, absorption, and extraction, but it has not been previously exploited in bioprocessing. In addition, the use of a flowing slurry dramatically reduces the pressure requirements for CCTC processes (maximum pressure of <15 psi) compared to that needed for packed column chromatography. The net result is that the entire flow path in CCTC is fully disposable, greatly facilitating the incorporation of CCTC in single-use bioprocessing facilities that can produce multiple products with rapid setup/changeover.
In contrast to multi-column systems, CCTC is a true steady-state process, with the resin flowing continuously through the static mixers-membrane modules that conduct the different chromatographic operations (Figure 2). CCTC completely eliminates the complex valve-switching operations that are characteristic of multi-column and simulated moving bed chromatography systems; the entire flow path in CCTC operates at steady state without any changes in flow rates or connections. This steady state operation not only facilitates process control and integration, but it also means that all of the resin in CCTC is in use at all times, leading to large increases in productivity relative to column chromatography. In addition, the pressure drop in CCTC is independent of the resin particle size, allowing the use of small particle size resins with faster binding kinetics and greater binding capacity. Recent data obtained using a prototype 20 to 25 µm particle size Protein A resin showed productivities of 140 g of antibody/L resin/hr, which is tenfold greater than that for a conventional packed column.8 The eluted product in CCTC is recovered at a lower concentration than in column chromatography since the maximum resin concentration in the flowing slurry is about 15% by volume, but this is readily accommodated by integrating a single pass tangential flow filtration (SPTFF) module with the elution step. SPTFF can not only increase the effectiveness of subsequent unit operations, but it can also facilitate the development of appropriate control strategies by providing an opportunity to adjust the product concentration in response to upstream disturbances in the operation of the perfusion bioreactor.
Figure 2: Flow path for resin (green line) and buffers in CCTC system.
Fully Integrated CCTC Process
Although most of the early work on CCTC was focused on initial product capture due to the very high cost of the Protein A chromatography step, CCTC can also be used for polishing operations in either bind-and-elute or flow-through mode. Recent studies have demonstrated a fully integrated continuous downstream process employing Protein A, anion exchange, and cation exchange CCTC steps for the purification of a monoclonal antibody. This three-step process was able to reduce host cell protein (HCP) concentrations from 690,000 ng/mg to less than 10 ng/mg (below the limit of quantification). The final product contained more than 99% monomer, comparable to and in most cases better than what was achieved by processes based on conventional column chromatography. CCTC can also be directly integrated with a perfusion bioreactor employing an appropriate cell retention device, with the clarified cell culture fluid used as the feed to a CCTC capture step based on Protein A. Cost projections suggest that a facility incorporating this type of fully integrated CCTC technology could produce 600 kg of monoclonal antibody per year with manufacturing costs of less than $40/g in a facility requiring only 5,000 square feet of manufacturing space.
Path Forward
The transition from batch to continuous processing was clearly identified back in 2011 in a presentation at the annual AAPS meeting by Dr. Janet Woodcock, at the time the Director of the Center for Drug Evaluation and Research at the FDA. Woodcock predicted “that manufacturing will change in the next 25 years as current manufacturing practices are abandoned in favor of cleaner, flexible, more efficient continuous manufacturing.” Robert Bradway, the CEO of Amgen, noted that “Today’s systems for producing drugs in bacterial or animal cells and then isolating them are hugely expensive and can take months. With more efficient processes in place, companies could swiftly increase production of drugs in high demand, and they could produce medicines for rare diseases more cost-effectively as well.”9
Although continuous operations have recently been introduced into several small molecule manufacturing processes, the adoption of fully continuous biomanufacturing has remained a largely theoretical concept. Much of the reason for this lies in the challenges associated with continuous downstream processing and, in particular, the development of an appropriate continuous technology for initial product capture. Continuous countercurrent tangential chromatography has a number of unique features that could address these challenges, enabling the development of low-cost, highly flexible continuous manufacturing of monoclonal antibodies as well as viral vectors for delivery of novel gene therapies. In combination with ongoing advancements in process analytical technology (PAT) for process monitoring and control, it may finally be possible to realize the vision for continuous biomanufacturing in the not-too-distant future.
References
- Badman C, Cooney CL, Florence A, Konstantinov K, Krumme M, Mascia S, Nasr M, Trout BL, Why we need continuous pharmaceutical and how to make it happen, J Pharm Sci, 108(11): 3521 (2019).
- Khanal O, Lenhoff AM, Developments and opportunities in continuous biopharmaceutical manufacturing, mAbs, 13(1): e1903664 (2021).
- Walther J, Godawat R, Hwang C, Abe Y, Sinclair A, Konstantinov K, The business impact of an integrated continuous biomanufacturing platform for recombinant protein production, J Biotechnol, 213: 3 (2015).
- Walther J, Lu J, Hollenbach M, Yu M, Hwang C, McLarty J, Brower K, Perfusion cell culture decreases process and product heterogeneity in a head-to-head comparison with fed-batch, Biotechnol J, 14: 1700733 (2019).
- Zydney AL, Continuous downstream processing for high value biological products. A review, Biotechnol Bioeng, 113(3): 465 (2016).
- Dutta AK, Tan J, Napadensky B, Zydney AL, Shinkazh O, Performance optimization of continuous countercurrent tangential chromatography for antibody capture, Biotechnol Prog, 32(2): 430 (2016).
- Zydney AL, New developments for membranes in bioprocessing -- A review, J Membr Sci, 620: 118804 (2021).
- Federenko D, Dutta AK, Tan J, Walko J, Brower M, Pinto NDS, Zydney AL, Shinkazh O, Improved Protein A resin for antibody capture in a continuous countercurrent tangential chromatography system, Biotechnol Bioeng, 117: 646 (2020).
- Weintraub K, Biotech firms in race for manufacturing breakthrough, MIT Technology Review (2013).
About The Author:
Andrew L. Zydney, Ph.D., is the Bayard D. Kunkle Chair and Professor of Chemical Engineering at The Pennsylvania State University. Zydney also serves as director of the Penn State site in the Membrane Science, Engineering, and Technology (MAST) Center. Professor Zydney's research is focused on the application of membranes in bioprocessing, including the purification of monoclonal antibodies, vaccines, and gene therapy agents. He is a recent recipient of the Alan S. Michaels Award for Innovation in Membrane Society and Technology (from the North American Membrane Society) and the ACS Separations Science and Technology Award (from the American Chemical Society). He served as editor-in-chief of the Journal of Membrane Science for more than 10 years and is past president of the North American Membrane Society (NAMS). Zydney serves on the board of directors for Chromatan Corp., a start-up company pursuing the commercialization of continuous countercurrent tangential chromatography for biomanufacturing.